Zircon and Zirconia Refractories
- kaasvin06127
- Aug 31, 2021
- 8 min read
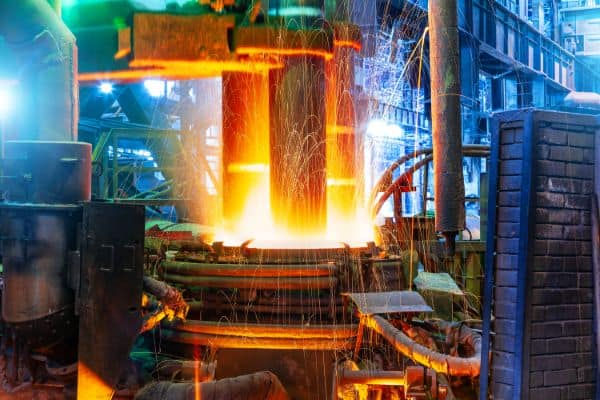
In conditions such as glass, fiberglass, or enamel furnaces, the resilience of refractory materials to heat stress and alkali corrosion is of great interest. In such conditions, refractory materials are commonly used to protect burners. Burner blocks, which are dedicated to this purpose, are subject to special limits that necessitate the use of high-performance materials. In contrast to silica and alumina, zirconia has a great resistance to alkali diffusion. This study focuses on refractory monoliths with high zirconia concentration in order to characterize the long-term corrosion of alkali resistant divisions. These complicated materials were tested in the lab to see how resistant they were to corrosion at temperatures ranging from 1300°C To 1400°C. Two exposure methods were utilized to confirm the results’ reliability: “covering self-crucibles” and “turning finger.” The refractory materials utilized in this investigation were exposed to high concentrations of sodium carbonate via a liquid or gaseous media. The findings highlight the interplay of sodium with the refractory’s numerous components. SEM and XRD were used to study the evolution of the microstructure and phases present in the refractory materials following the sodium assault. The role of silica-based phases in refractory degradation is explored, as well as the mechanisms of alkali diffusion inside the refractory and microstructure change. The methodology utilized in the study was corroborated by observations made in the field on a used burner block.
Refractory materials, such as alkali elements, which are added to the batch as melting agents, corrode in glass, fiberglass, and enamel furnaces because they evaporate in the furnace atmosphere. In both the liquid and gaseous phases, these melting agents interact with the refractory materials. The corrosion and hardening of the bricks in direct contact with the glass bath, as well as the bricks that make up the furnace superstructure and are in touch with gaseous corrosive chemicals, received a lot of attention. This research focuses on burner blocks, which are subjected to unique limitations in high-temperature furnaces, particularly oxy-fuel furnaces, and have received little attention. The burner block is designed to shield the burner from the high temperatures of the furnace. It is formed of refractory material and is an integral aspect of the burner’s construction, since it leads the combustion fluids (oxidant and fuel) through the last few centimeters of the furnace. A running burner block is depicted in this diagram. The burner block faces significant thermal gradients depending on its design, as the temperature of injecting the reactants is lower (up to 1500°C lower) than the temperature of the hot combustion gases, and the flame root is generally anchored at a short distance from the burner block (1 to 100 cm), which heats the block. Depending on the burner operating parameters, the injection velocity of the reactants changes the recirculation patterns at the burner block, and the recirculation zones specific to the burner neighborhood continuously renew the atmosphere at the block surface, bringing high temperature corrosive agents. For burner block applications, high performance refractory materials are required due to these unique restrictions. The goals of this research are to provide a process for selecting refractory materials for our burner blocks and to figure out how silicon bounded material and alumina are destroyed. Several common materials were subjected to alkali corrosion in both liquid and gaseous forms, and their relative resistance was assessed. The materials’ names are not disclosed in this study due to confidentiality concerns. Their composition and microstructure were examined before and after corrosion testing, providing information on the mechanisms of corrosion and guiding the selection of appropriate materials for burner block applications.
In igneous environments like granitic pegmatites and syenite, as well as metamorphic environments like schists and gneiss, zircon occurs naturally as an accessory mineral. It appears in the host rock as square, prismatic crystals or grains, which are usually tiny and widely dispersed. Zircon is not generally suited to economic utilization in this form (concentrations rarely surpassing 1 wt percent). Weathering activities, combined with zircon’s natural inclination to separate from regular silica sand due to its high density1, have resulted in the creation of huge secondary deposits of zircon sand in river and beach placers with increased concentrations. Other heavy minerals commonly found in these deposits include ilmenite and rutile (both titanium containing minerals), monazite (a phosphate of rare earth metals and thorium), garnet, staurolite, and kyanite. Zircon may also be found in tin or copper-bearing deposits. Zircon has evolved from a low-value by-product of the mining and processing of heavy mineral sands for the extraction of the titanium minerals ilmenite and rutile since the 1960s. Heavy mineral sand deposits contain 1–10% heavy minerals on average. Gravity separation is used to obtain a concentration that contains more than 95 percent heavy minerals. Magnetic and electrostatic separation procedures extract zircon from the complex mineral mix, as well as other valuable minerals such as ilmenite, rutile, and monazite. Due to the presence of minerals other than zircon, especially monazite, these operations are associated with high radioactive concentrations, necessitating the use of considerable radiation protection measures. The lack of these significant radioactive concentrations when the zircon is separated from these minerals results in a substantially lower radiological risk. The processing of heavy mineral sands up to and including the separation of zircon is not addressed within the scope of this report in order to focus only on the risks associated with the processing and usage of zircon. However, Annex I has a brief description of the method for completeness. The majority of zircon production is shipped in bulk, unpackaged, from the country of origin via seagoing bulk carriers. A shipment is usually 1000 t, although it can be up to 10,000 t. Zircon can be transported by rail or road unpackaged in volumes of a few tens of tons per conveyance, or packaged in 1–2 t bulk bags or on pallets of generally 25 bags weighing 40 kg each. Individual pallets or containers of palletized zircon are transported.
The density of zircon is approximately 4690 kg/m3 (range: 4200–4800 kg/m3). It is a very robust and resistant mineral that has been undisturbed by the Earth’s surface’s natural, low-temperature processes. It has a low chemical reactivity, a melting point well above 2000oC, a high refractive index (1.80–1.98), and a Mohs hardness of 7–7.5, making it harder than quartz (which has a Mohs hardness of 7) but softer than corundum and diamond (with Mohs harnesses of 9 and 10, respectively). Size of the particles According to reports, commercial zircon sand has a median diameter of 110-130 µm and a rather narrow size range, with little material smaller than 75 µm or bigger than 250 µm. The average particle size is between 100 and 200 µm. The particle size of zircon sand must be decreased by milling for many uses, resulting in intermediate products such as zircon flour and micronized zircon. Since the 1980s, the amount of zircon sand treated into smaller particle sizes before usage has expanded dramatically, fueled by the ceramic industry’s strong demand for zircon in this form, and more than 70% of all zircon sand is now processed in this fashion.
The density of zircon is approximately 4690 kg/m3 (range: 4200–4800 kg/m3). It is a very robust and resistant mineral that has been undisturbed by the Earth’s surface’s natural, low-temperature processes. It has a low chemical reactivity, a melting point well above 2000oC, a high refractive index (1.80–1.98), and a Mohs hardness of 7–7.5, making it harder than quartz (which has a Mohs hardness of 7) but softer than corundum and diamond (with Mohs hardnesses of 9 and 10, respectively). Size of the particles Commercial zircon sand has a median diameter of 110-130 µm and a narrow size range, with little material smaller than 75 µm and greater than 250 µm. Particle sizes typically range from 100 to 200 µm. The particle size of zircon sand must be decreased by milling for many uses, resulting in intermediate products such as zircon flour and micronized zircon. Since the 1980s, the amount of zircon sand treated into smaller particle sizes before usage has expanded dramatically, fueled by the ceramic industry's strong demand for zircon in this form, and more than 70% of all zircon sand is now processed in this fashion. Baddeleyite scattered through magnetite bearing and apatite bearing zones in magnetite ores with 0.15 percent ZrO2. The purity of the product is comparable to that of the highest commercial grade previously produced in South Africa, but it contains less radiation. Because commercial sources of natural zirconia are few, 80–90% of the zirconia used in industrial applications is synthetic.
High strength, high fracture toughness, outstanding wear resistance, high hardness, excellent chemical resistance, good oxygen conductivity, and extremely good refractory qualities are all characteristics of zirconia that are useful for industrial applications. In advanced technology applications in the electronics industry, zirconia also has qualities that are significant for the formulation of ceramics with piezoelectric, pyroelectric, and dielectric properties. The monoclinic, tetragonal, and cubic phases of zirconia have distinct crystal shapes. The monoclinic phase is stable until it reaches roughly 1200°C, when it transitions into the tetragonal phase. The tetragonal phase is stable up to 2300°C, after which it undergoes a further change to the cubic phase. Up to the melting point of 2680°C, the cubic phase occurs. The monoclinic to tetragonal phase transition is accompanied by an 8% fast volume shift that reverses on cooling, limiting the use of pure zirconia in dense refractory applications due to the risk of structural failure. Zirconia in its pure state is known as stabilized zirconia because of these phase transitions. % ZrO2, 0.05 % SiO2, 0.15 % TiO2, 0.01 % Fe2O3, and 0.02 % SO3 are typical compositions of unsterilized zirconia used in particular ceramic applications requiring high purity. Cubic oxides — typically magnesium, calcium, or yttrium oxides — can be added to monoclinic zirconia during manufacturing to prevent this disruptive volume shift. This results in stabilized zirconia with a cubic solid solution structure that does not phase change from room temperature to melting point. It has a consistent thermal expansion curve and excellent refractory qualities. To generate a fully stabilized zirconia, more than 16 mol % (8 wt %) CaO, 16 mol % (6 wt %) MgO, or 8 mol % (14 wt %) Y2O3 must be added to the zirconia structure. A transformation hardened material known as partially stabilized zirconia (PSZ) is created when the stabilizing oxide is introduced at a concentration lower than that necessary for complete stabilization in the cubic phase. At temperatures below 1000°C, this lesser amount of stabilizer leads the pure zirconia’s structure to become a mixture of cubic, tetragonal, and/or monoclinic phases (with the tetragonal phase being the dominant one), and a pure tetragonal phase above 1000°C. PSZ is also known as tetragonal zirconia polycrystal (TZP) or tetragonally stabilized zirconia because of this. PSZ typically contains at least 8 mol % (3 wt %) MgO, 8 mol % (4 wt %) CaO, and 3–4 mol % (5–7 wt %) Y2O3.
Manufacture of considerable quantities of two essential zirconium chemicals: ZOC (produced from zirconium hydroxide reaction followed by hydrochloric acid dissolution) and zirconium tetrachloride (derived from the high temperature chlorination of zircon). Although both have applications as chemical products in and of themselves, their primary function is as processing intermediates. Zirconium tetrachloride is used to make zirconium metal, while zirconium oxide is utilized to make high purity zirconia and other zirconium compounds. Zirconium basic carbonate (ZBC), acid zirconium sulphate tetrahydrate (AZST), zirconium basic sulphate (ZBS), ammonium zirconium carbonate (AZC), potassium hexafluorozirconate (KFZ), and zirconium acetate are some of the other zirconium compounds manufactured in considerable amounts (ZRA). Smaller quantities of more specific zirconium compounds are generated. Antiperspirants, paint driers, and paper coatings are just a few of the applications for zirconium compounds. Additional information Because of zircon’s chemical inertness, the majority of the uranium and thorium contained within it was present during the mineral’s crystallization from molten host rock (although some may also occur within other minerals present as inclusions in the zircon sand grains, for example monazite). The decay components of uranium and thorium are bonded inside the zircon crystal structure, replacing a small number of zirconium atoms. The uranium atoms are not bound within the crystalline matrix in most other uranium-containing minerals, including uranium ores, but instead constitute part of the cementing material between the grains. Because of the nature of the zircon crystal, it is difficult to remove uranium and thorium without destroying the crystal lattice. 9 Acid leaching studies performed on various zircon sand samples, including milled samples, over a range of pressures, temperatures, and oxidizing conditions have shown that no substantial amounts of uranium or thorium are leached from the zircon material, even under the most hostile conditions. Future research investigations on zircon sand revealed that the amount of 210Po released at 1200oC is minimal, despite the fact that this temperature is substantially higher than the volatilization temperature of 210Po (100–150oC) and even higher than its boiling point (962oC). Only at considerably higher temperatures, such as those seen in electric arc furnaces, does the crystal structure break down, allowing the volatilization of 210Po and other radionuclides to occur.
Comments